Learning Objectives
- Define the concept of a fitness trade-off with respect to survival and reproduction, and evaluate a tradeoff graph to explain why organisms cannot simultaneously maximize survival/lifespan and reproduction.
- Define and explain the “mutation accumulation” hypothesis for how the seemingly maladaptive process of aging evolved, and predict the types of genes that could cause aging under the mutation accumulation hypothesis of aging.
- Know that apoptosis, or programmed cell death, is a normal part of development and regulation of tissues and organs in multicellular organisms, give examples for development and maintenance.
- Define and give examples of senescence of cells and organisms.
- List and explain the stages of decomposition of a multicellular organism.
Senescence represents age-related decline in fitness
[Modified from Khan Academy]
Senescence is the age-related decline in an organism’s ability to survive and reproduce. As soon as an organism reach reproductive maturity, it begins to senesce.
All living things need energy and nutrients to grow, maintain their bodies, and reproduce. In nature, these resources are in limited supply, and there is often competition for access to them (e.g., to sunlight and minerals for plants or food sources for animals). Thus, each organism will have non-infinite resources to divide among activities like survival (growth, body maintenance) and reproduction.
What does it mean for an organism to allocate its limited resources “well” in this context? From an evolutionary standpoint, it means that the resources are distributed among the potential activities (growth, maintenance, reproduction) in a way that maximizes fitness, or the relative number of offspring the organism leaves in the next generation. Organisms with inherited traits that cause them to distribute their resources in a more effective way will tend to leave more offspring than organisms lacking these traits, causing the traits to increase in the population over generations by natural selection [2,3].
Over very long periods of time, this process results in species with a collection of life history traits, like number of offspring, timing of reproduction, amount of parental care, etc. These traits are well-adapted for their role and environment. The optimal life history strategy may be different for each species, depending on its traits, environment, and other constraints [2].
That limitation on how energy resources are allocated over a lifetime doesn’t match the perfect world in which an organism would be “immortal and highly fecund,” meaning it would live forever and produce many rounds of offspring, because the more kids you put into the population, the more influence your genes have in future generations (Darwinian fitness). We never see that pattern in nature, and that’s actually a good thing or the earth would be overrun with organisms, and we would have already have run out of resources.
In the real world, organisms experience constraints between growth and reproduction (see figure).
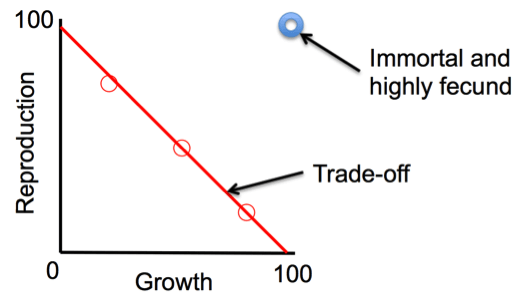
The “ideal” immortal and highly fecund organism does not exist in nature. Instead, the red circles represent trade-offs between high growth, or survival, and high reproduction. Most species fall along the red line. (Image credit: Chrissy Spencer, CC-BY)
The “ideal” immortal and highly fecund organism does not exist in nature. Instead, the red circles represent trade-offs between high growth, or survival, and high reproduction. Most species fall along the red line.
Below, we’ll examine some tradeoffs in life history strategies and see examples of plants and animals that use strategies of different types. For example, plants like coconuts and chestnuts have low fecundity, meaning they produce small numbers of seeds, but each seed is energy-rich and has a good chance of germinating into a new organism. Plants with high fecundity, such as orchids, take the opposite approach: they usually make many small, energy-poor seeds, each of which has a relatively low chance of surviving.
In animals, we also see the tradeoff of many offspring accompanied by low investment/parental care. A typical sea snail (whelk) produces hundreds of eggs at a pop, and these eggs hatch to yield baby snails that are pretty self-sufficient from the get-go. In fact, the baby snails in the first 10%, percent of eggs that hatch will enthusiastically eat their slower-hatching siblings for breakfast! [4]
Accumulation of deleterious mutations could explain why we age
These survival and fecundity tradeoffs have been hypothesized to lead to aging! Here’s how that might happen: Imagine you have some genes that are expressed later in life, after you begin reproducing, such as the genes that cause Alzheimer’s or low calcium fixation that increases the risk of bone fractures. If your life history strategy evolves to be live fast, die young, then those later in life genes never become your phenotype because they never get expressed. Mutations that arise in those genes cannot be purged by natural selection acting against them because selection never sees them. Why is that? Selection only acts on phenotypes, not on genotypes, so if a gene is never expressed, its phenotype is never around for selection to act against. In this way, certain genes can accumulate mutations that are never removed from the population by natural selection, so they persist. This mutation accumulation would mean that, should those organisms happen to live a little longer, they will express mutated genes that might cause poor function, disease, or even early death.
Apoptosis and the end-replication problem of telomeres
[Modified from Khan Academy]
Certain cells, perhaps including those that express mutated genes, can be removed by the body’s own regulation through a process called programmed cell death. For example, have you ever wondered how your fingers formed? It turns out that the cells between your developing fingers were instructed to die long ago, while you were still an embryo. If they hadn’t done so, you would have webbed hands, or perhaps just paddles of tissue with no fingers at all.
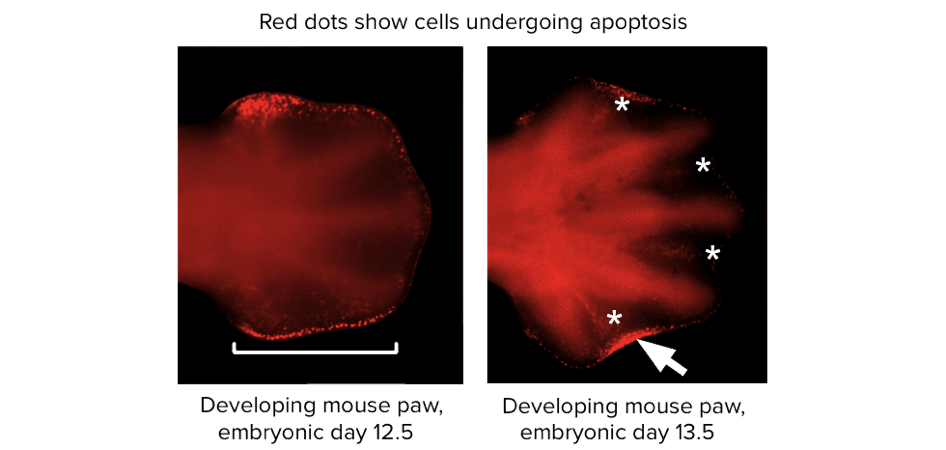
Microscope images from a scientific paper, showing a developing mouse paw. The cells between the developing digits are stained by a marker that indicates apoptotic cells. Image modified from “Duplication of digit 4 is preceded by reduced apoptosis and expanded chondrogenesis in the posterior limb mesenchyme,” by Farin et al. [2], CC BY 4.0.
Cells that undergo apoptosis shrink and deform, while the DNA in the nucleus gets chopped up into small pieces. In the end, the entire cell splits up into small chunks, each neatly enclosed in a package of membrane. What happens to the chunks? They release signals that attract debris-eating (phagocytic) immune cells, such as macrophages, that come and digest them.
Many cells in the human body have the built-in ability to undergo apoptosis (in the same way that they have the built-in ability to copy their DNA or break down fuels). Basically, apoptosis is a general and convenient way to remove cells that should no longer be part of the organism.
- Some cells need to be “deleted” during development:
- to whittle an intricate structure like a hand out of a larger block of tissue,
- loss of a tadpole’s tail as it turns into a frog,
- removal of unneeded neurons as neural circuits in the brain are “wired.”
- Some cells are abnormal and could hurt the rest of the organism if they survive, such as cells with viral infections or DNA damage.
- Cells in an adult organism may be eliminated as part of housekeeping, to make way for new cells or remove cells needed only for temporary tasks.
So, apoptosis could help us consider another hypothesis about why organisms age: our somatic cells are mortal, with perhaps only 40-60 replications in them before they die. This “disposable soma,” where parts wear out over time and cease to function properly, implies that a finite energy amount over a lifetime must be shared between growth/metabolism, reproduction, or repair/maintenance of parts (cells, organs, systems). It turns out that the reason for apoptosis in older somatic cells is related to a problem with DNA replication for linear chromosomes.
Unlike bacterial chromosomes, the chromosomes of eukaryotes are linear, meaning that they have ends. These ends pose a problem for DNA replication. The DNA at the very end of the chromosome cannot be fully copied in each round of replication, resulting in a slow, gradual shortening of the chromosome. Why is this? When DNA is being copied, it relies on short bits of primer DNA to help get the new DNA started. The last short bit at the end of a chromosome doesn’t have anywhere for the primer to land—it’s off the end of the DNA! So, each cell division causes the DNA in the daughter cells to get a little bit shorter.
To prevent the loss of genes as chromosome ends wear down, the tips of eukaryotic chromosomes end in specialized, highly repetitive extra DNA called telomeres.
The repeats that make up a telomere are eaten away slowly over many division cycles, providing a buffer that protects the internal chromosome regions bearing the genes, at least for a while. Telomere shortening has been connected to the aging of cells because the progressive loss of telomeres frays the ends of the DNA. In some cells, that fraying triggers a DNA damage system called p53 (more on that when we cover cancer) that halts the cell cycle to allow DNA repair. In many cell types, the shortened (damaged) DNA cannot be repaired, which may explain why cells can only divide a certain number of times [4]. Germ cells have a special enzyme (telomerase) that rebuilds the telomeres in egg and sperm cells, so new zygotes have full length chromosomes without shortened ends.
Telomerase is active in germ cells (i.e. sperm and eggs) and some adult stem cells, but it’s not usually active most somatic cells (cells of the body). Interestingly, many cancer cells have shortened telomeres, and telomerase is active in these cells. Perhaps if telomerase could be inhibited by drugs as part of cancer therapy, their excess division (and thus, the growth of the cancerous tumor) could potentially be stopped.
Cancer prevention is a good reminder of why it’s not always a bad thing for cells in your body to die. It’s actually important that some cells of our bodies do die – not randomly, but in a carefully controlled way.
Is there any way to halt the aging process?
Halting or even reversing aging would generate immortality, much discussed in fiction and fantasy, and the object of western European explorers in search of the fountain of youth, among others.
Ideas for slowing aging include caloric restriction, enhancing cell-repair and detoxification mechanisms like enzymes that remove reactive oxygen molecules from cells. We’ll discuss some research ideas on extending lifespan in class.
Here’s a video on senescence in cells, including telomere’s, the Hayflick limit, and apoptosis:
and here’s the SciShow follow up on aging:
Death
Organisms do eventually die. When multicelluar organisms die, nearby microorganisms ramp up activity in a process called decomposition. Decomposition is the breakdown of the organism by bacteria and fungi into the simple building blocks of which the organism was made. The stages of decomposition—fresh, bloat, active decay, advanced decay, and dry (or remains)—are explained in the video and image below.