Learning Objectives
- Identify autotrophs and heterotrophs and know how they acquire energy and matter.
- Know that bioenergetics chemistry using ATP, redox reactions, and chemiosmosis is universal in all living organisms on earth.
- Explain two phosphorylation mechanisms that cells use to gain energy in the form of ATP.
- Follow the paths of electrons and hydrogen ions to establish proton gradients to synthesize ATP.
Metabolic energy for life ultimately comes from the sun [Adapted from Openstax Biology]
The living cells of every organism constantly use energy. Nutrients and other matter are imported, metabolized (broken down), synthesized into new molecules, and transported around the cell and the entire organism. For example, the large proteins that make up your muscles are actively built from smaller molecules. In contrast, complex molecules like carbohydrates are broken down into simple sugars that the cell uses for energy. Just as energy is required to both build and demolish a building, energy is required for both the synthesis and breakdown of these molecules.
Energy for this work inside living organisms comes either directly from the sun for autotrophs, also called photosynthesizers. These include plants, lots of bacteria and some archaea. Organisms that cannot photosynthesize are called heterotrophs, and they gain energy from eating other living organisms. When one organism eats another, some of the energy it has stored in sugars in its tissues is transferred to the consumer. Scientists call this transfer or flow of energy through a living system bioenergetics. Bioenergetics describes energy flow through all types of living systems, from cells to ecosystems, such as one in the figure below.
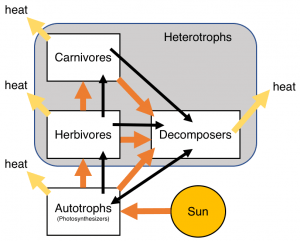
Energy flows through the different organisms in an ecosystem (orange arrows) and is lost as heat (yellow arrows). Matter, such as carbon, nitrogen and other nutrients, cycles around the ecosystem (black arrows).
Energy flows from the eaten organism to the consumer with an average 90% energy loss or waste of energy to heat and decay by decomposers. By contrast, storing up chemical energy from sunlight through photosynthesis is much more efficient, though lots of sunlight energy goes unused by photosynthesizers.
Once energy is stored inside a cell, stepwise chemical reactions build up and break down complex molecules. Some of these chemical reactions are spontaneous and release energy, whereas others require energy to proceed. Just as living things must continually consume food to replenish what has been used, cells must continually produce more energy to replenish the energy used by the many chemical reactions that constantly take place. The sum of all the chemical reactions that occur inside a cell, including those that use energy and those that release energy, are the cell’s metabolism.
Metabolism breaks down carbohydrates
The metabolism of sugar (a simple carbohydrate) is a classic example of the many cellular processes that use and produce energy. Living things consume sugar as a major energy source because sugar molecules have a great deal of energy stored within their bonds. The breakdown of glucose, a simple sugar, is described by this chemical equation:
It looks pretty straightforward, but glucose breakdown involves a series of complex chemical reactions called glycolysis and the citric acid cycle. We say that a cell that is breaking down sugars using these reactions is respiring, or undergoing respiration. Most living organisms respire, and the exceptions use fermentation to break down carbohydrates.
Photosynthesis builds carbohydrates
A few organisms, namely plants and many bacteria and archaea, can run chemical reactions that are the opposite of respiration. They use photosynthesis to store captured energy in the chemical bonds of carbohydrates (sugars). During photosynthesis, plants use the energy of sunlight to convert carbon dioxide gas (CO2) into sugar molecules, like glucose (C6H12O6). Glucose synthesis is described by this equation, which should look strangely familiar because it’s the reverse of the previous equation!
Because the process of photosynthesis makes a larger, energy-storing molecule, some energy has to be spent. The energy currency of all cells is a very high-energy molecule called ATP, or adenosine triphosphate. Just as we use dollars as currency to buy goods, cells use molecules of ATP as energy currency to perform immediate work. The sugar (glucose) is stored as either a starch or a glycogen chain of many smaller glucose molecules. Energy-storing chains like these are broken down into glucose, which can be processed to supply the cell with molecules of ATP to do work.
As you can see in the image below, ATP is a small, relatively simple molecule with the potential for a quick burst of energy in the chemical bonds between the beta and gamma phosphate groups.
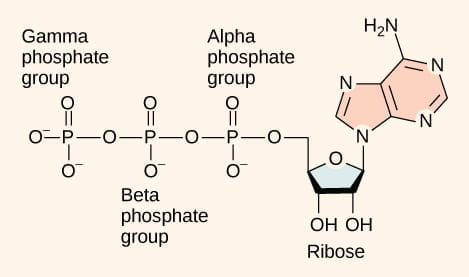
Adenosine triphosphate, or ATP, is a small sugar that serves as the primary energy currency of the cell, storing chemical energy in the bonds between the beta and gamma phosphate groups [Image credit: Openstax Biology]
The reverse reaction ofADP+Pi+free energy→ATP+H2O regenerates ATP from ADP + Pi. Cells rely on the regeneration of ATP just as people rely on the regeneration of spent money through some sort of income. ATP regeneration requires an input of free energy.
Energy Metabolism is universal in life on earth [Adapted from Jung Choi]
Now that we know the key elements of bioenergetics, we can explore the energetic processes fundamental to all living cells. Scientists hypothesize that the processes explored below were likely present in the last universal common ancestor (LUCA) of all life on earth.
All cells – Bacteria, Archaea, Eukarya – use the energy released via ATP hydrolysis to ADP and inorganic phosphate to perform most work inside the cell. How do cells make ATP? Cells can regenerate ATP from ADP in either of two ways: either by substrate-level phosphorylation or by oxidative phosphorylation.
Substrate-level phosphorylation means that a phosphate is transferred to ADP from an organic compound (the substrate). A few enzymes in the glycolysis and citric acid cycle reactions make ATP through substrate-level phosphorylation. However, these reactions tap only a small fraction of the potential energy in glucose, so only a small amount of ATP is made by substrate-level phosphorylation in cells undergoing respiration. Substrate-level phosphorylation by glycolysis enzymes is the major source of ATP only in cells undergoing fermentation (fermentative cells perform glycolysis, but no respiration). All other organisms use a much more efficient type of phosphorylation, described next.
Oxidative phosphorylation is an apparently more complex process where a proton-motive force across a membrane powers an ATP synthase enzyme complex (a molecular machine) to make ATP from ADP and inorganic phosphate. Oxidative phosphorylation allows a cell to release large amounts of free energy and makes the vast majority of the ATP in respiring cells. Surprisingly, oxidative phosphorylation appears to be at least as ancient as glycolysis and fermentation, and operates in environments both with and without oxygen.
The energy for ATP synthesis via either substrate-level phosphorylation or oxidative phosphorylation comes from organic molecules (such as sugars and carbohydrates), or from sunlight, or from inorganic electron donors. Metabolic chemical reactions capture energy from these various sources (organic compounds, sunlight or chemicals) and couple them to synthesis of ATP from ADP.
Cellular respiration results when chemical redox reactions transfer electrons
In metabolic reactions, the most free energy is released by chemical reactions known as redox reactions. Redox reactions transfer electrons between molecules. A molecule that gains electrons is reduced; a molecule that loses electrons is oxidized, which is how “redox” gets its name. You can use the phrase “OIL RIG” to remember that Oxidation Is Loss, Reduction Is Gain. Different molecules have different tendencies to gain electrons, called the redox potential. A redox reaction between a pair of molecules with a large difference in redox potential results in a large release of free energy.
Cellular energy metabolism features a series of redox reactions. Heterotrophs oxidize (take electrons from) organic molecules (food) and give those electrons to an electron carrier molecule, called NAD+ (in the oxidized form) that accepts electrons from food to become NADH (the reduced form). NADH then cycles back to NAD+ by giving electrons to (reducing) an electron acceptor protein in a membrane, thus becoming oxidized to NAD+ again. In the membrane, the electrons are transferred down an electron transport chain, consisting of a series of membrane proteins and molecules with increasing redox potential. Components of the electron transport chain use the sequential releases of free energy to pump protons across the membrane against their electrochemical gradient.

The electron transport chain, part of cellular respiration, transfers electrons from donors to acceptors,operating in the cell membrane. The process generates an gradient of protons, in the form of hydrogen ions, outside the membrane. [Image modified by C. Spencer from a Wikimedia image by Fvasconcellos in the public domain.]
Cellular respiration is the cascade of electrons transfers (redox reactions) that culminates in the reduction of the terminal electron acceptor. The amount of energy released by these redox reactions, and thus the amount of energy available for ATP synthesis, depends on the redox potential of the terminal electron acceptor. Oxygen (O2) has the greatest redox potential, and thus respiration in an oxygen-rich environment like earth’s current environment results in the most ATP synthesized. Bacteria and Archaea (and some Eukarya) can use other terminal electron acceptors with lower redox potential when oxygen is not available; type of respiration produces less ATP.
Chemiosmosis forms ATP using a proton gradient
All bacteria and archaea maintain a proton gradient (pH gradient) across their plasma membranes. The interior of the cell is relatively alkaline, whereas the exterior is relatively acidic. A 1000-fold difference in proton concentrations across the membrane results in a proton motive force, consisting of both the chemical concentration gradient of protons and a voltage gradient across the membrane. Free energy is released by the return of protons across the membrane down their concentration and voltage gradients. The cell membrane is relatively impermeable to protons, so energy released by diffusion of protons via transport proteins and proton channels can be used by the cell to accomplish work, such as active transport of substances across the membrane and synthesis of ATP from ADP and inorganic phosphate. The proton diffusion-powered synthesis of ATP is called chemiosmosis.
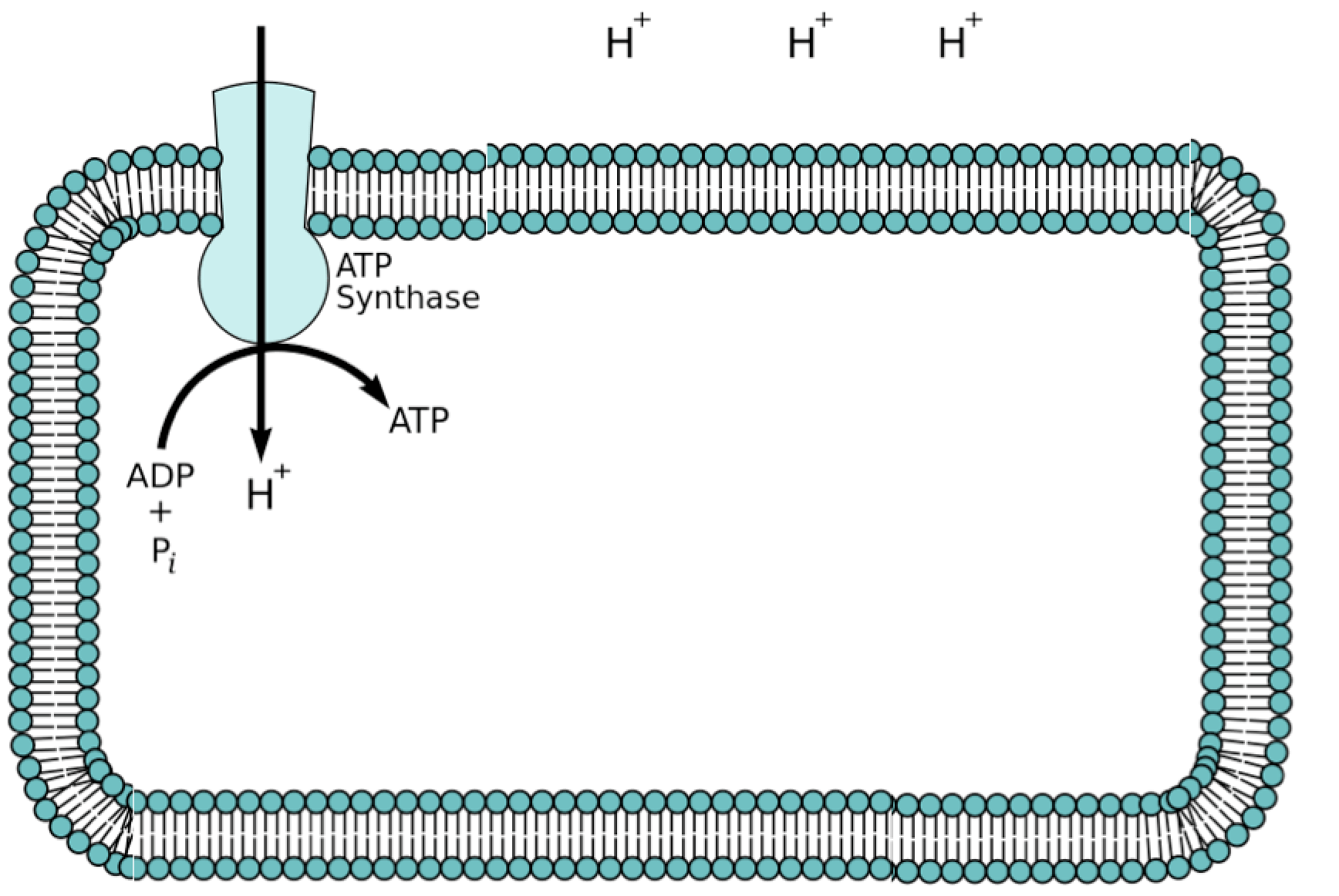
The ATP synthase is an ion channel that runs through the membrane. It uses the hydrogen ion gradient as a proton motive force to power the formation of ATP from ADP and inorganic phosphate. [Image modified by C. Spencer from a Wikimedia image by Fvasconcellos in the public domain.]
The ATP synthases in mitochondria, chloroplasts, and bacteria are all structurally similar, and highly homologous at the molecular sequence level (Watt et al. 2010). Archaeal ATP synthases are less closely related but similar. The generation of a proton gradient across a membrane and chemiosmosis are universal to life on earth, and are fundamental ways for cells to make a living. Lane and colleagues speculate that “proton power” may have been the earliest form of energy metabolism, essential to, and pre-dating, the last universal common ancestor, LUCA (Lane, 2009; Lane et al. 2010).
References:
Lane, N 2009 Was our oldest ancestor a proton-powered rock? New Scientist 19 October 2009 http://www.newscientist.com/article/mg20427306.200-was-our-oldest-ancestor-a-protonpowered-rock.html?page=1
Lane, N, JF Allen, W Martin 2010 How did LUCA make a living? Chemiosmosis in the origin of life. BioEssays DOI 10.1002/bies.200900131
Watt, IN, MG Montgomery, MJ Runswick, AGW Leslie, JE Walker 2010 Bioenergetic cost of making an adenosine triphosphate molecule in animal mitochondria PNAS 107 : 16823-16827 doi:10.1073/pnas.1012260107