Learning Objectives
- Define and recognize a population as a group of interacting individuals who are members of the same species, a community as a set of species that interact with each other, and an ecosystem as organisms in a community interacting with each other and with the non-living components in their environment, such as lakes, rivers, mountains, soil, rainfall, etc.
- Know that four factors matter for the growth of a population of members of the same species: births and immigration increase population size, while deaths and emigration decrease population size.
- Compare and distinguish between exponential and logistic population growth equations and interpret the resulting growth curves.
- Define life history traits and identify patterns of life history traits that correspond to exponential versus logistic population growth patterns.
- Know the named detrimental (–) / beneficial (+) interactions, including that predation, parasitism, and herbivory are enemy/victim interactions, and recognize examples of them.
- Name and recognize ecosystems and the biotic (living) and abiotic (non-living) factors that impact where and how organisms live.
- Identify how climate (temperature and precipitation) affects the habitat locations and ranges of biomes.
- Explain biomagnification of toxins through non-linear accumulation of the toxins in higher trophic levels of a food chain.
- Recognize food webs as the feeding interactions between species and predict the outcome if a species in a food web disappears or increases dramatically in population.
Population ecology
A population is a group of interacting organisms of the same species. Most populations have a mix of young and old individuals. Quantifying the numbers of individuals of each age or stage gives the demographic structure of the population. In addition to demographic structure, populations vary in total number of individuals, called population size, and how densely packed together those individuals are, called population density. A population’s geographic range has limits, or bounds, established by the physical limits that the species can tolerate, such as temperature or aridity, and by the encroachment of other species. Population ecologists often first consider the dynamics of population size change over time, of whether the population is growing in size, shrinking, or remaining static over time.
Basic Dynamics – Exponential Population Growth
The most basic approach to population growth is to begin with the assumption that every individual produces two offspring in its lifetime, then dies, which would double the population size each generation. This population doubling at each generation is how an ideal bacterium with unlimited resources would reproduce.

When resources are unlimited, populations exhibit exponential growth, resulting in a J-shaped curve. Source: OpenStax Biology
Because the births and deaths at each time point do not change over time, the growth rate of the population in this image is constant. Although the growth rate is fixed with time, the population doesn’t grow linearly in this model because every individual that was born in that generation reproduces. As a result, the population explodes in size very quickly—exponentially in fact. In nature, a population growing at this dramatic rate would quickly consume all available habitat and resources. In actuality, natural populations have size limits created by the environment.
Logistic Population Growth levels off at a carrying capacity
To consider how resource limitation affects population growth, we need to incorporate the concept of carrying capacity, the maximum population size that the environment can sustain. Any individuals born into this population would increase the population size unless the number of deaths balanced or outnumbered births. If the population size remains the same from one generation to the next, then individuals must also be dying at a similar rate. With exponential population growth, the population growth rate was constant. With the addition of a carrying capacity imposed by the environment, the logistic population growth rate slows as the population size increases, and growth is zero when the population reaches carrying capacity.

When resources are limited, populations exhibit logistic growth. In logistic growth, population expansion decreases as resources become scarce, and it levels off when the carrying capacity of the environment is reached, resulting in an S-shaped curve. Source: OpenStax Biology
Population size is regulated by factors that are dependent or independent of population density
Biological and non-biological factors can influence population size. Biological factors include interspecific interactions like predation, competition, parasitism, and mutualism, as well as disease. Non-biological factors are environmental variables like temperature, precipitation, disturbance, pollution, salinity, and pH. All of these factors can change population size, but only the biological factors (except mutualism) can “regulate” a population, meaning they push the population to an equilibrium density, or carrying capacity. Of the biological factors, mutualism does not regulate population size because mutualisms promote population increase through beneficial interactions with another species.
The biological factors of competition, predation, etc. that regulate population growth affect dense versus sparse populations differently. For instance, communicable disease does not spread quickly in a sparsely packed population, but in a dense population, like humans living in a college residence hall, disease can spread quickly through contact between individuals. Density plays a key role in population regulation in the following ways:
- Territoriality: Maintaining a territory will enable an individual to capture enough food to reproduce, where space is a limiting resource.
- Disease: Transmission rate often depends on population density
- Predation: Predators may concentrate on the most abundant prey
- Toxic Wastes: Metabolic by-products accumulate as populations grow
Identifying evidence of density regulation requires a field or lab experiment that manipulates density and quantifies the response in population growth. Often an (easier to measure) proxy of population growth, like survival or reproductive output, stands in as a quick metric of the births and deaths that will impact population growth. The characteristic negative correlation in the image below is evidence of density-dependent population regulation: higher densities yield lower survival.

Van Burkirk and Smith (1991) manipulated salamander (Ambystoma laterale) larval density in the field and found lower salamander survival in the high density treatments on North Government Island, Isle Royale, Michigan. (Image after van Burkirk and Smith. 1991. Ecology 72(5): 1747-1756.)
Here’s Hank Green’s take on Population Growth to help you review these ideas:
Life history traits and their evolution
Individuals in a population experience a life cycle of birth, growth and development, maturity to adulthood, and then decline into reproductive senescence. How energy is allocated to these different aspects of the organisms survival is called their life history, and that energy allocation generates characteristic life history traits, traits that impact survival and reproductive output: size at birth, age at maturity, size at maturity, number and size of offspring (fecundity), reproductive value, lifespan and senescence, which we will define as the decline in fecundity with age. Life History Theory explains how evolution optimizes these survival and reproductive characteristics in different populations, affecting parameters such as: how big and fast individuals grow, when they reach sexual maturity, how many offspring they have each time they reproduce, how many times they reproduce, and when they die.
Notice that survival and reproduction are “optimized,” not maximized. This is because when evolution increases one of these traits, say survival of the parent, the result is usually a decrease in some aspect of reproduction, such as number of offspring produced each year, and vice versa. This optimization generates a life-history trade-off, depicted as a negative relationship between survival and reproduction (see figure below).
A life-history trade-off, depicted as a negative relationship between survival and reproduction, is probably caused by energy allocation split between survival and reproductive output for an organism.
The leading hypothesis for trade-offs in survival and reproduction is that energy is the limiting factor: organisms have finite energy, so if they allocate energy toward survival, then they don’t have as much available to reproduce. As a result, some organisms like the Chinook salmon reproduce only once in their short lifetime, while others such as Atlantic Cod—and humans—reproduce many times.
Selection for life history trade-offs between reproduction and survival can be related to a population’s growth rate and the environment’s carrying capacity.
- Populations that grow exponentially tend to have life history traits that maximize growth rate (live fast, die young). These populations include traits like having very large numbers of offspring, relatively small body size, early sexual maturity, broad dispersal of offspring, limited parental care, and short life-span. Species include insects, bacteria, many types of plants, and some small mammals such as rodents. These species tend to be found in places that are not limited by carrying capacity. While they tend to produce many, many offspring, each individual offspring has a low chance of survival. Their population sizes can change dramatically over short periods of time.
- Life history traits that are selected for in organisms living at densities near the environmental carrying capacity have traits opposing traits: relatively small numbers of offspring, comparatively large body size, late sexual maturity, offspring which require a high degree of parental care, and longer life-span. These species include large organisms such as many large mammals, some species of reptiles, and some species of birds. In the absence of natural disaster, disease epidemic, or other catastrophic event, the population size of these species tend to remain relatively stable and close to the maximum carrying capacity.
Species Interactions
Interspecific interactions can be classified as beneficial, detrimental, or neutral for one or both species in the interaction, like this:
Competition, where two species use the same limited resource and thus are in conflict with each other over that resource, can be confused as an enemy-victim relationship; however, it doesn’t fit the definition because both species are harmed rather than one benefiting.
Enemy-victim interspecific interactions have a negative impact on one species while providing benefits to the other. The three types are predator-prey interactions, parasite-host interactions, and herbivory. These are all win-lose situations. Parasitism, but not predatory or herbivory relationships, is a type of symbiosis.
In the parasite-host interaction, also called parasitism, one species (the parasite) lives on or in another species (the host) that it harms over time, sometimes even resulting in the death of the host. Parasitism is a win for parasite and a loss for the host.
Another win-lose scenario is predator-prey interactions, or predation. Predation involves one species (the predator) consuming—yes, eating—the other (the prey), which obviously dies in the exchange. A well-studied example is the lynx-hare interaction in the Canadian arctic.
Herbivory is similar to predation in the sense that a grazer eats a plant. However, some grazing interactions can be argued to provide a benefit because grazing can promote the growth of new plant material. To determine if an herbivore-plant interaction is a win-lose, the researcher needs to measure the cost and benefit to the plant.
Finally, mutualism is a type of symbiosis where both species benefit in the interspecific interaction. For example, plants have fungi that live on or in their roots, called mycorrhizae. The fungus gains access to sugars like glucose and sucrose from the root system, and the plants use the phosphorus and nitrogen that the fungi “fix,” or process into a biologically usable form. Without this fairly universal mutualism in almost all plants, we would not have crops to eat or as much oxygen to breathe.
Ecosystem ecology
An ecosystem is a collection of interacting “biotic” or living elements and “abiotic” or non-living elements. Abiotic factors strongly influence where and how organisms live on Earth. Globally, rainfall and temperature are the abiotic factors that define the Earth’s biomes. Notice on the terrestrial biome map below that the equator has wet biomes, like tropical wet forest, while the bands 30 degrees north and south contain the world’s great deserts, among them the Sahara in the northern hemisphere and the deserts of Australia.
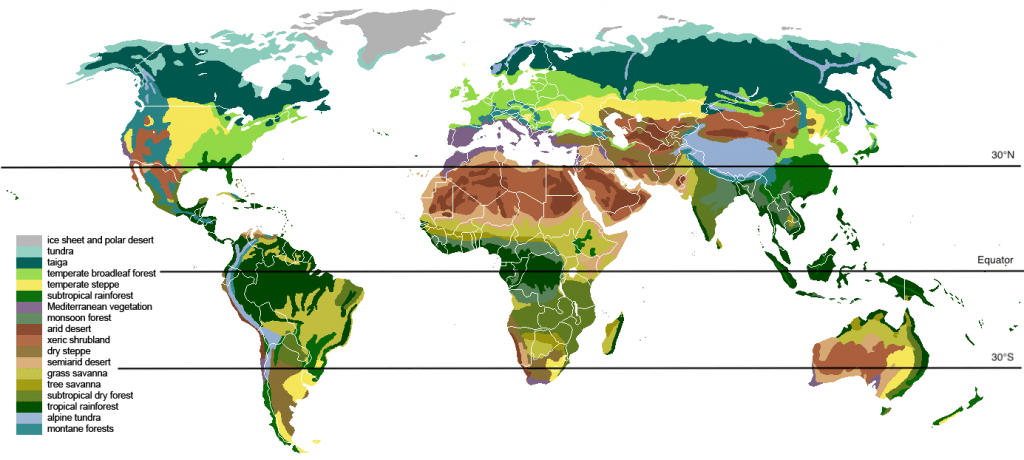
The main biomes of the world. Modified from image by Ville Koistinen (CC BY-SA 3.0, 2007).
This map of biomes allows us to see global ecosystem patterns, such as how deserts or forests are organized latitudinally. Interruptions to this pattern occur when major geologic features run counter to latitude. For example, in western South America the biomes run north-to-south instead of east west. The shift is caused by the high-elevation Andes mountain range, which runs along the western coast and disrupts east-west patterns. The mountains’ height disrupts the east-to-west climate patterns more readily evident in Africa and Australia.
If we plot biomes along axes of temperature and precipitation, then we can use graphical organization to predict how environmental changes can alter the biome found in a specific location.
Some common biomes graphed by temperature and precipitation. (Image source: commons.wikimedia.org/wiki/File:PrecipitationTempBiomes.jpg)
For instance, if a wet tundra biome experiences an increase in average annual temperature, what biome would you predict that location to shift to over time?
In biomes governed by water, precipitation matters less while temperature and winds take on a more dominant role. One example of this is ocean upwelling, depicted in the figure below.
In the figure, ocean upwelling recycles nutrients in the ocean. Wind (upper/green arrows) blows offshore and displaces surface waters. Waters from the ocean bottom (lower/red arrows) move to the surface in a process called upwelling, bringing with them nutrients from the ocean depths. Why are there nutrients at the bottom of the ocean? When ocean dwelling organisms die, they sink to the bottom, sequestering to the bottom of the ocean all the nutrients, such as chemical elements, their bodies contain: carbon, nitrogen, oxygen, hydrogen, etc.
Unlike the sunlight energy that powers the heat-engine of the planet, the nutrients on Earth are finite and reusable. Nutrients like phosphorous, nitrogen, and even carbon are the matter on the planet, and this matter cycles through biotic and abiotic locations. For example, in the ocean in the figure above, carbon is in the living organisms, and when those organisms die that carbon falls to the ocean bottom, where biotic activity in the absence of sunlight is much reduced compared to near the surface. Upwelling can bring the carbon back into biotic circulation. Alternatively, the carbon can remain at the bottom and eventually become incorporated into abiotic sedimentary rock. The carbon in rock will be out of circulation until erosion, geologic activity, or human activity like drilling or mining brings it to the surface.
Nutrients can cycle between living organisms when organisms eat each other. Predation or herbivory (enemy-victim interaction) is an obvious way to gain energy for organisms that cannot make their own energy via photosynthesis from the sun. We can depict the movement of energy gained from enemy-victim interactions in a diagram called a food chain, seen below.

A simple terrestrial food chain shows how energy moves from the eaten to the eater as a rabbit eats grass, and then is eaten by a hawk. Each level of consumption is called a trophic level and is labeled by the species trophic function in the ecosystem (CC-BY by C. Spencer.)
In a food chain, the direction of the arrows indicate the direction of the flow of energy. Most species feed on a variety of prey and plants—obviously a hawk is able to catch other prey in addition to rabbits, and those other prey, say mice, could also eat grass. So, the simple food chain above is oversimplified, and in fact the concept of a food chain itself is too simple for most ecosystems. Instead, a web of feeding patterns better reflects the ways that most species interact in an ecosystem. The basic food web shown below includes the grass-rabbit-hawk food chain, as well as other species at the three trophic levels.
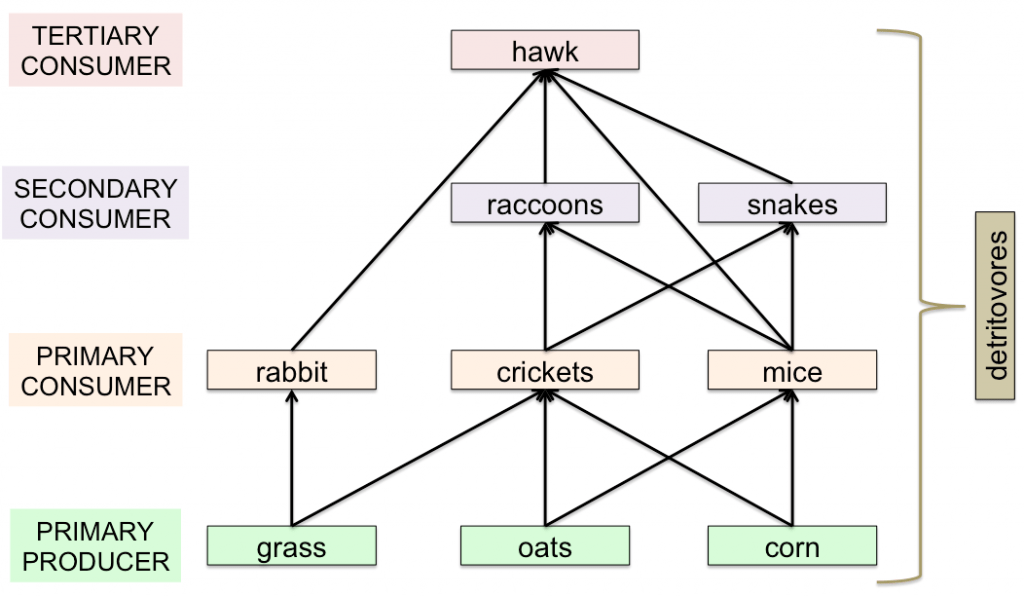
A simple terrestrial food web, where several species occupy each trophic level in a community of interactions. All of the organisms would also have an arrow flowing to detritivores, which are the decomposers of dead organic matter in the ecosystem. (Image Credit: Chrissy Spencer, CC-BY.)
As with the food chain, the arrows show the direction of energy transfer. Every species should also have an additional arrow flowing to the detritivores to indicate the flow of energy upon death (those arrows aren’t shown but are indicated by the bracket). Ecologists use food webs to ask about dependencies between species. In the diagram above, if the hawk population becomes critically small, what impact would that have on the rest of the ecosystem?
Organisms gain energy through food web dynamics, but there is a potentially detrimental environmental consequence of eating others in contaminated environments: biomagnification. Biomagnification is the increase in concentration of persistent, toxic substances in organisms at each trophic level, from the primary producers to the top consumers. While organisms are very inefficient at acquiring energy through food (on average 10% of the energy is available to the eater), all of the toxins are retained by the eater, often stored in fat or tissue, or trapped in the liver or kidneys. Individual organisms accumulate toxins over their lifetimes.
In the 1940s, a synthetic insecticide called DDT (full name: dichloro-diphenyl-trichloroethane) was developed and deployed to reduce insect populations, malarial risk, and to protect crops. DDT use had unintended consequences. It bioaccumulated in birds that ate those insects. Birds with high DDT levels laid thin eggs, which caused high bird offspring mortality. As a result of the work of biologist and writer Rachel Carson and others, the use of DDT was banned in the United States in the 1970s. Other substances that bioaccumulate and biomagnify are heavy metals like mercury, lead, and cadmium, as well as PCBs (full name: polychlorinated biphenyls). PCBs were banned in 1979 in the US from use in coolant liquids. The figure below shows an example of PCB biomagnification in the Saginaw Bay of Lake Huron:
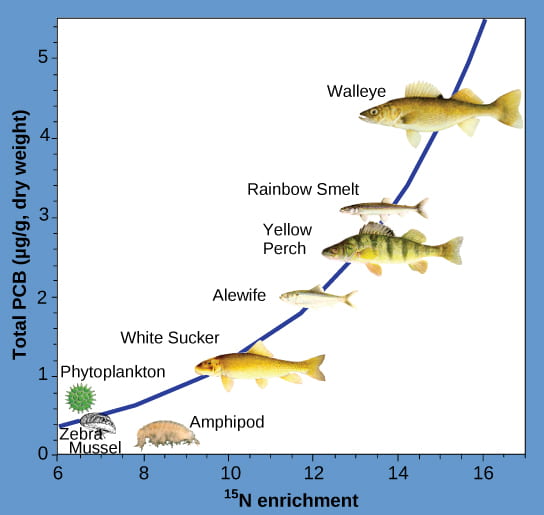
Fish in the higher trophic levels accumulate more PCBs than those in lower trophic levels. PCB concentrations increase more than the linear expectation (shown by tracking naturally occurring heavy isotopes of nitrogen) for accumulation when moving up trophic levels in the Saginaw Bay ecosystem of Lake Huron. The faster-than-linear increase is called biomagnification. (credit: Patricia Van Hoof, NOAA, GLERL)
In class, we’ll consider what could happen to the ecosystem if biomagnification or other changes to the food web occur.
Hank Green’s Crash Course on Ecosystem Ecology summarizes these topics in a quick 10 minute lecture:
Ideas and text on this page include content from Open Stax Biology and the BioPrinciples online textbook at bio1510.biology.gatech.edu.
Literature Cited
Van Burkirk and Smith. 1991. Ecology 72(5): 1747-1756.